Molecular Dynamics Simulation Service
Creative Proteomics provides cutting-edge molecular dynamics simulation services to study molecular interactions, structural dynamics, and thermodynamics. Utilizing platforms like AMBER, GROMACS, NAMD, and CHARMM, we offer tailored solutions for protein-ligand interactions, protein-protein dynamics, structural optimization, and solvent effects, supporting drug discovery and biomolecular research with precision and efficiency.
Submit Your Request Now
×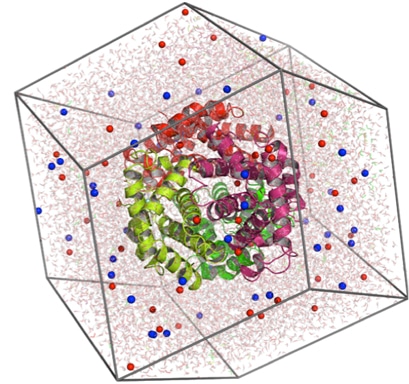
Jochen S. Hub, et al. Spontaneous Quaternary and Tertiary T-R Transitions of Human Hemoglobin in Molecular Dynamics Simulation. PLoS Comput Biol 2010,6(5): e1000774
- Define
- What We Provide
- Research Areas
- Technology Platforms
- FAQs
- Case Study
What is Molecular Dynamics Simulation?
Molecular Dynamics (MD) Simulation is a computational technique that uses principles from classical mechanics and statistical physics to model and predict the dynamic behavior of interacting particles—atoms and molecules—over time. By applying Newton's equations of motion to molecular systems, MD simulations offer invaluable insights into molecular conformational changes, structural stability, and thermodynamic properties under predefined conditions, such as specific temperatures, pressures, or solvent environments.
Unlike static models from NMR or X-ray crystallography, MD simulations dynamically account for protein flexibility and conformational changes—key aspects for studying biological phenomena like transport, molecular recognition, enzyme catalysis, and allosteric regulation. This capability makes MD simulation an essential tool in drug discovery, where it helps identify cryptic or allosteric binding sites, predict binding affinities, and understand protein-ligand interactions.
Types of Molecular Dynamics Simulation Methods We Provide
Coarse-Grained Dynamics Simulation:
This method simplifies molecular models, making it ideal for large systems like membranes or multiprotein complexes over extended timescales. By reducing computational complexity, coarse-grained simulations allow researchers to observe overall dynamic behaviors for large biomolecular assemblies over longer periods.
All-Atom Molecular Dynamics (MD) Simulation:
This approach retains atomic-level details, making it ideal for studying precise intermolecular interactions. All-atom simulations are essential for analyzing ligand-receptor interactions, protein conformational shifts, and calculating binding free energies, particularly useful in drug discovery and structural optimization.
Replica Exchange Molecular Dynamics (REMD):
REMD enhances sampling by simulating multiple replicas of the system at different temperatures, allowing the system to cross local energy minima. This method is well-suited for investigating conformational changes in complex molecular systems, especially at extreme temperature conditions.
Steered Molecular Dynamics:
This technique applies external forces to guide the system along a specific path, allowing for the study of reaction pathways or mechanical properties. It is especially useful for observing specific molecular events like ligand dissociation or receptor activation and for understanding the structural responses and mechanical stability of biomolecules.
Umbrella Sampling Simulation:
Umbrella sampling uses biased potentials to enhance sampling across different states, supporting precise free energy calculations. This method is ideal for analyzing binding free energies and specific transition states, often applied in molecular recognition studies.
Targeted Molecular Dynamics:
This approach simulates conformational transitions, providing insights into structural changes critical to biological functions, such as protein folding, enzyme activation, and signal transduction. Targeted MD is valuable for understanding functional shifts in molecular structure.
Post-Simulation Analysis Services:
These services offer in-depth analysis of MD simulation results, extracting key dynamic and thermodynamic insights. Key analyses include RMSD/RMSF for stability, hydrogen bond analysis, solvent-accessible surface area (SASA), principal component analysis (PCA), and binding free energy calculations (MM-PBSA/MM-GBSA). These tools provide a comprehensive understanding of molecular behavior and interaction mechanisms.
Research Areas in Molecular Dynamics Simulation
Self-Assembly Simulations
Self-assembly simulations model the spontaneous organization of molecules into structured arrangements, such as micelles, vesicles, or nanoparticle coatings. These simulations are essential for understanding the self-organization processes seen in biological membranes, drug delivery systems, and nano-material fabrication, providing insights into both the driving forces and final structures that emerge under physiological conditions.
Protein-Ligand Interactions
Simulating the interactions between large biomolecules and smaller compounds, such as drugs or ligands, allows for the detailed study of binding mechanisms and affinities. This is particularly valuable in drug discovery, where MD simulations reveal binding sites, interaction energies, and the role of protein flexibility in binding, enabling targeted drug design and optimization.
Protein-Protein Interactions
MD simulations of protein-protein interactions offer atomic-level insights into complex formation, stability, and binding specificity. These simulations are instrumental in studying signaling pathways, immune responses, and multi-protein complexes by helping to identify critical contact regions and binding hot spots that regulate biological function.
Solvent-Solute Interactions
Simulations of solvent-solute interactions allow researchers to study the influence of the solvent environment (such as water or organic solvents) on molecular behavior and stability. By analyzing how solvent molecules interact with solutes, these simulations provide information on solubility, structural stability, and potential aggregation effects.
Optimization of Macromolecular and Small Molecule Structures
Structure optimization simulations refine the 3D conformations of macromolecules, such as proteins, nucleic acids, and small molecules, through energy minimization and molecular dynamics equilibration, to achieve energetically favorable structures. This refinement process ensures that models used in further simulations are biophysically relevant and representative of in vivo conditions, improving the accuracy of predictions regarding molecular interactions.
Structure-Function Relationships
Simulations investigating the structure-function relationship analyze how structural changes within a molecule affect its biological function or physicochemical properties. These studies are essential for understanding enzyme catalysis, molecular recognition, and conformational changes that drive biological activity.
Technology Platforms for Molecular Dynamics Simulation
1. AMBER
Widely recognized for its precision in studying organic molecules and small proteins, AMBER allows simulation under various physiological conditions and is instrumental in studying the interactions between small molecules and proteins.
Applications: Suitable for exploring protein folding, molecular binding, and conformational stability.
2. GROMACS
Highly efficient for simulating complex biological macromolecules, such as proteins, lipids, and nucleic acids.
Applications: Useful for a broad range of applications including protein-ligand binding studies, membrane dynamics, and large-scale biomolecular simulations.
3. NAMD
Known for its scalability, NAMD is capable of simulating large macromolecular systems with high performance, making it ideal for studies involving DNA, RNA, and protein complexes.
Applications: Particularly suited for investigating the conformational dynamics and structural interactions of large biomolecules under varied conditions.
4. CHARMM
Supports various molecular mechanics and MD simulation types, including periodic boundary conditions and thermodynamic integration for free-energy calculations.
Applications: Effective for studying allosteric regulation, protein folding, and biomolecular flexibility.
FAQs
How do you ensure that the MD simulations accurately reflect real experimental conditions?
We carefully set up each MD simulation to closely mimic experimental conditions. This includes using precise force fields, ensuring proper temperature and pressure controls, and selecting relevant solvent environments. Additionally, we validate simulation results against available experimental data, like NMR or crystallographic data, to ensure that our models are representative. Adjustments can be made during the simulation process to refine accuracy as needed, based on any feedback or new data you provide.
How are force fields selected, and can I specify a preferred force field for my project?
Force field selection depends on your system's specific requirements and the intended outcome of the simulation. For instance, AMBER force fields work well for organic molecules and proteins, while CHARMM is preferred for membrane systems and protein-ligand binding. We are flexible with force field selection, and if you have a preferred choice or specific requirements, we can accommodate these preferences. Each force field is evaluated for its suitability in achieving the most reliable results for your particular project.
How is data analyzed post-simulation, and what insights can I expect from the analysis?
Post-simulation analysis is tailored to your research objectives, offering both basic and advanced insights. Standard analyses include RMSD/RMSF for structural stability, hydrogen bond interactions, and solvent accessibility (SASA). Advanced insights may include free energy calculations (MM-PBSA/MM-GBSA), which reveal binding affinity and interaction strengths, and principal component analysis (PCA) for understanding collective motions. Our analysis reports offer a comprehensive view of dynamics, interaction patterns, and structural transitions relevant to your biological questions.
What's the advantage of using Replica Exchange Molecular Dynamics (REMD) in simulations?
REMD is particularly useful for overcoming energy barriers that can trap molecular systems in local minima, especially when studying complex conformational changes or folding pathways. By running simulations at multiple temperatures, REMD allows for better sampling of the conformational space, revealing states that might otherwise be inaccessible. This method is particularly advantageous in protein folding studies, as it helps reveal less favorable but biologically relevant conformations that contribute to a protein's functional dynamics.
Can MD simulations help predict the stability of mutant proteins or altered biomolecules?
Yes, MD simulations are highly effective for assessing the stability of mutant proteins. By simulating mutants at physiological conditions, we can observe changes in folding, stability, and flexibility. This approach helps in understanding how specific mutations impact structural stability, potential aggregation, or functional alterations. We provide comparative analyses between wild-type and mutant forms, which is valuable for understanding disease-related mutations or optimizing protein design in synthetic biology.
How are steered molecular dynamics (SMD) and umbrella sampling different in studying binding/unbinding events?
Steered MD (SMD) and umbrella sampling offer complementary insights into binding/unbinding. SMD applies a controlled external force to guide the system along a pathway, making it ideal for studying unbinding events, like ligand dissociation, where an external trigger is involved. Umbrella sampling, on the other hand, introduces a biased potential to enhance sampling of transition states and is better suited for free energy calculations of binding interactions over multiple states. Depending on your research objective, we recommend one or both methods to provide a thorough understanding of binding events.
How can MD simulations assist in drug discovery, specifically in identifying allosteric sites?
MD simulations help in identifying allosteric sites by revealing conformational flexibility and cryptic binding sites that may not be visible in static structures. By analyzing the protein's dynamics, we can detect transient binding pockets that arise due to natural motions, providing potential targets for allosteric modulators. Techniques like REMD and targeted MD are particularly useful for observing conformational shifts that expose these cryptic sites, offering new avenues for drug discovery beyond traditional active-site targeting.
What is the maximum system size that can be simulated, and are there limitations on simulation timescales?
System size and timescale are primarily constrained by computational power. Using coarse-grained MD, we can simulate larger systems, such as entire viral particles or cellular membranes, over extended timescales (microseconds to milliseconds). For all-atom MD, system size is typically limited to a few hundred thousand atoms for realistic timescales. Advances in hardware and simulation techniques allow for efficient scaling, and we offer guidance on balancing detail and scale for optimal results.
How do solvent conditions in MD simulations impact molecular behavior, and can specific solvents be simulated?
Solvent conditions play a critical role in stabilizing structures and influencing interactions in MD simulations. We can simulate both water (polar) and organic (nonpolar) solvents, depending on the molecular system and environment. For example, simulating membrane-bound proteins may involve lipid bilayers, while drug solubility studies can utilize organic solvents. By accurately modeling these environments, we offer insights into solubility, aggregation tendencies, and structural stability in real-world conditions.
Are hybrid quantum mechanics/molecular mechanics (QM/MM) simulations available for highly reactive systems?
Yes, we offer QM/MM simulations for systems requiring atomic-level accuracy, such as enzyme-catalyzed reactions or metalloprotein studies. QM/MM divides the system into quantum (QM) and molecular (MM) regions, allowing for accurate modeling of reactive sites while retaining computational efficiency. This hybrid approach is ideal for studying bond-breaking/forming events, catalytic mechanisms, and electronic effects in biomolecules, providing a more detailed look at chemical reactivity in biological systems.
Learn about other Q&A.
Case Study
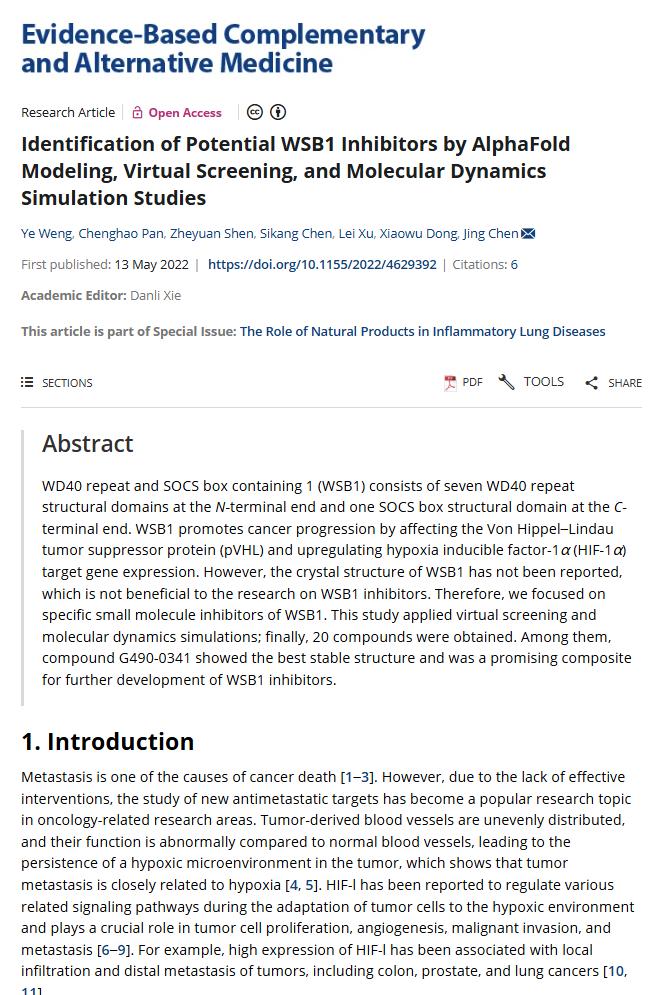
Identification of Potential WSB1 Inhibitors by AlphaFold Modeling, Virtual Screening, and Molecular Dynamics Simulation Studies
Journal: Evidence-Based Complementary and Alternative Medicine
Published: 2022
- Background
- Materials & Methods
- Results
- Reference
The study focuses on identifying inhibitors of the WD40 repeat and SOCS box-containing protein 1 (WSB1), which is known to promote cancer progression by influencing hypoxia-inducible factor-1α (HIF-1α) expression. WSB1 has been shown to drive tumor metastasis, making it a critical target for cancer treatment. Due to the lack of a known crystal structure for WSB1, researchers utilized AlphaFold2 to model WSB1's structure, followed by molecular dynamics simulations to identify stable binding compounds, aiming to discover novel small-molecule WSB1 inhibitors.
- Protein Structure Modeling:
AlphaFold2 was employed to predict the structure of WSB1, followed by molecular dynamics simulations to refine and stabilize the model. This included a 200 ns simulation to remove structural inconsistencies.
- Protein-Peptide Docking:
The Maestro and GlideSP tools were used for molecular docking, with additional structural preparation via MOE's quick prep tool. Ligand preparation involved defining WSB1's active pocket and docking optimization to identify high-affinity binding poses.
- Virtual Screening:
A screening campaign on ChemDiv's 1.6 million compound database was conducted using AutoDock-GPU, GlideSP, and other methods to narrow down top compounds. The screening process involved multiple stages of scoring and filtering to improve binding accuracy.
- Binding Pose Metadynamics:
Desmond's binding pose metadynamics simulations were applied to evaluate ligand stability within the binding pocket, focusing on hydrogen bond retention and binding mode stability using PoseScore and PersScore.
AlphaFold2 Protein Structure Prediction:
Since no crystal structure of WSB1 was available, the study utilized AlphaFold2 to predict its structure. AlphaFold2, a deep learning-based model, provided a highly accurate, atomic-level structural prediction for WSB1. Researchers used this model as a foundation for subsequent molecular docking and simulation studies. The predicted structure was refined and validated with molecular dynamics (MD) simulations, reaching equilibrium after 25 ns, which resulted in a stable conformation with minimal fluctuations.
Molecular Dynamics Simulation of WSB1:
The 200 ns MD simulations repaired structural irregularities in the AlphaFold-predicted WSB1 model, reaching equilibrium with root mean square deviation (RMSD) stabilizing below 1 Å. The structural analysis showed 6.67% α-helix and 34.64% β-strand content, indicating specific secondary structural elements essential for binding interactions.
WSB1 Docking with Ligands:
The study focused on exploring WSB1's binding interactions with ligands, especially thyroid-hormone-activating type 2-iodothyronine deiodinase (D2), an associated protein. Docking simulations indicated that WSB1 had a strong binding affinity for the D2 peptide, particularly through an 18-amino acid loop that positioned D2 close to the WSB1 binding site. Critical residues, including Arg174, Arg315, and Tyr218, were involved in these protein-protein interactions, stabilizing WSB1's function as an E3 ubiquitin ligase for D2.
Virtual Screening:
Using a library of 1.58 million compounds from the ChemDiv database, a multi-stage virtual screening identified compounds with potential binding affinity for WSB1. After initial scoring and refinement, the process narrowed down to 20 candidate molecules. These compounds underwent additional analyses to evaluate binding poses and identify potential WSB1 inhibitors.
Binding Pose Metadynamics:
The binding stability of the selected compounds was further analyzed with binding pose metadynamics (BPMD) simulations, which provided insights into the relative stability and hydrogen bond persistence of each binding mode. The study found that compound G490-0341, which formed stable interactions with WSB1 residues Asp175, Arg315, Tyr276, and Trp38, exhibited the highest stability. Metadynamics simulations confirmed G490-0341's advantage over other compounds, as it showed strong binding to hydrophobic residues, essential for WSB1 inhibition.
Conformational Analysis of Leading Compounds:
In-depth analyses of four top compounds (G490-0341, G610-0188, Y043-6168, Y044-5019) revealed stable binding through hydrogen bonds and hydrophobic interactions with key WSB1 residues. Among them, G490-0341 showed the strongest binding, involving key residues such as Arg315 and Asp175, suggesting its significant potential as a WSB1 inhibitor.
Reference
- Weng, Ye, et al. "Identification of potential WSB1 inhibitors by AlphaFold Modeling, virtual screening, and molecular dynamics simulation studies." Evidence‐Based Complementary and Alternative Medicine 2022.1 (2022): 4629392.